Atomically Resolved Dynamics
Former Research Group - R. J. Dwayne Miller
The group is devoted to understanding the primary processes of molecular reaction dynamics with a major emphasis on understanding the connection between the chemistry driving biological functions and the highly optimized structures that have evolved in biological systems. To this end, we have developed some of the world’s brightest electron sources to literally light up atomic motions to directly resolve this structure-function correlation and to provide the most fundamental (atomic) basis for understanding chemical and biological processes.
Our group represents a broad range of cultures, languages and backgrounds, and as our photos suggest, we're a pretty active bunch.
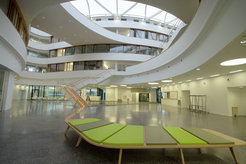
The group members are housed in the Center for Free-Electron Laser Science (CFEL), located on the DESY DESY campus. This unique environment brings together the expertise and technical capabilities gained from high energy physics with a particular emphasis on structural determination. The group has four femtosecond high brightness electron beam lines covering electron energies from low energy (kV) to high energy nonrelativistic sources (100 kV), to the relativistic regime (5 MeV). This range of capabilities allows the group to study isolated quantum systems in the gas phase, surface reaction dynamics, to solution phase reaction dynamics, with atomic resolution. In addition, the group has built up new capabilities in the UV to open up broadband multidimensional UV spectroscopy with a particular emphasis on protein and DNA dynamics. The materials development effort is also housed here, along with the theory subgroup. These subgroups are charged with developing new materials specifically to probe various reaction mechanisms/structural transitions and to help guide experiments, respectively.
What do we do?
The research focus in on understanding molecular reaction dynamics on the fastest possible time scales, with an overall objective of bringing new insight into the structure-function correlation to chemical and biological processes. A number of new spectroscopic tools have been developed (coherent multidimensional spectroscopies, laser technologies) to track the quantum state dynamics. Information on both electronic and vibrational degrees of freedom can be extracted from this approach. However, reaction dynamics are governed by mostly strongly coupled modes to the reaction coordinate and these tend to be low frequency, strongly damped modes that are not amenable to spectroscopic methods. The only prospect for truly mapping out reaction dynamics is to directly observe atomic motions on the primary timescales. The group is unique in that we developed electron source technology of sufficient brightness to literally light up atomic motions to enable veritable atomic movies of the primary processes involved in structural transitions. We are striving to further increase the source brightness to enable atomic level movies of more and more complex systems with a direct resolution of the structure-function correlation in biological systems as the ultimate goal. This objective will provide the most fundamental (atomic) level basis possible for understanding biological functions at the molecular level. We are getting close.
Chemical and the biological processes involve hundreds to thousands of atoms. How can we discern the relevant degrees of freedom? For complex systems, correlations to specific structures or spatial relationships of interacting atoms are quickly lost due to stochastic fluctuations and collisions. By going to extremely short times scales to study the very fastest processes, i.e. the primary processes, the atomic correlations are conserved and a detailed atomic level description can still be retrieved. We are just staring to learn from atomically resolved reaction dynamics that there is an enormous reduction in dimensionality at critical points (barrier crossing or within the transition state region) to just a few key modes. This new insight holds promise to change how we think about chemistry. It may be possible to characterize reaction mechanisms in terms of these reaction modes or power spectra much like vibrational spectra to characterize equilibrium fluctuations. This new insight came about by the group’s drive to resolve the structure-function correlation with respect to how proteins work. Early studies found evidence for the involvement of collective modes in directing chemical processes into functions (Genberg et al Science 1991), which led to the development of the Collective Mode Mechanism (Miller, Acc. Chem. Res 1994, Miller, Can J Chem 2001) to explain the functionally relevant motions of proteins. But what are the modes? Are there conserved structural motifs that lead to certain classes of chemistry being exploited in biology? We feel there is a kind of periodic table waiting to be discovered for biology but it is not based on elemental composition but on topologies. There are clearly very similar structural motifs that are used over and over again in nature that border on mathematical descriptions of topologies. There is no other prospect to answer these questions, and to look for the inherent correlations in biological structures, than to directly observe the functionally relevant motions at the atomic level of detail. A decision was made back in 1989 to invest heavily in source development to achieve atomically resolved structural dynamics (with complementary efforts to track electronic degrees of freedom or quantum state dynamics as required to fully understand a problem using optical perturbative methods to trigger the dynamics).
The enormity of the challenge at the time can be appreciated. One of the “holy grails” in chemistry has been to directly observe the atomic motions driving chemical processes. This objective was thought to be outside the realm of direct experimental observation. We would always have to rely on indirect information to infer the key motions to reconstruct reaction pathways. This thinking was based on the extraordinary space-time resolution one would have to achieve to be able to capture chemistry in action. The relevant timescales are in the 10 to 100 femtosecond timescale (1 femtosecond = 10-15 seconds). This extremely fast time scale was only in its infancy back in 1989 but it is now readily accessible with the latest developments in solid state lasers that can readily achieve near single cycle optical pulses of a few femtoseconds. The other consideration is spatial resolution. To image atomic motions one needs an Å and preferable sub-Å wave source. This limits the source selection to either hard x-rays or high energy electrons with pulse durations on the 100 fs timescale or shorter for femtosecond pump-probe or stroboscopic methods of recording atomic motions. It is no problem to generate femtosecond few electron or x-ray photon pulses to give atomic resolution – for static images after sufficient averaging. The problem is that the very process of triggering the chemistry to play out its reaction course changes the system. The real challenge to achieve atomic resolution to molecular dynamics is the source brightness (you just get one look). One has to have a source with sufficient brightness to literally light up atomic motions on the femtosecond time scale.
The first atomic movie of a structural transition on the prerequisite space-time resolution was accomplished by the group (Siwick et al, Science 2003) using a new concept in ultrabright electron source generation. This was the first subpicosecond time resolved diffraction studies with sufficient diffraction orders resolved to invert to real space structural dynamics. Prior to the group’s work it was thought that it was thought that it would be impossible to generate high brightness electron pulses for imaging atomic motions due to the inherent electron-electron coulombic repulsion or space charge effects that would broaden the pulse. New insight into the electron pulse propagation dynamics came from an effectively exact solution to the coupled equations of motion of some 10,000 electrons (sufficient for single shot atomic resolution of simple unit cells in diffraction) that the electrons do not lose space-time correlation (Siwick et al JAP 2002). The electrons as the front of the pulse stay at the front and the electrons at the back stay at the back and ultimately form a near perfect linear chirp. Two means of generating ultrabright electron pulses for atomic imaging were immediately apparent from this work, i.e. compact electron guns to minimize pulse broadening and pulse compression methods exploiting the linear chirp. The first atomic movie was captured with the compact gun electron gun design and is still the most robust source for atomically resolved dynamics. The first work of this kind focused on arguably the simplest structural transition of melting albeit under very special conditions that gave us our first glimpse at the atomic level of the long predicted homogeneous nucleation phenomena. The surprise was that it was possible to drive the system from solid to liquid so fast that nucleation could be arrested to as few as ten atoms. This singular observation led to a new concept in laser surgery (Amini-Nik et al, PLoS 2010) that avoids explosive nucleation growth and cavitation induced shock wave damage that causes massive collateral damage in laser surgery. This new approach is the first method by any means to achieve surgery with no scar tissue formation, and with single cell precision with respect to borders. In effect, the long held promise of the laser to achieve minimally invasive surgery has been achieved and without excessive fibroblast formation leading to scar tissue. This connection is made as this development came from basic science, a strong desire to observe the atomic details of structural transitions. It literally came out of the blue (blue sky research). The laser has been around for over 50 years. The prospect of minimally invasive surgery was immediately recognized but collateral damage to surrounding tissue has always been an issue. A strictly application based approach did not solve the problem. It required an atomic level perspective of laser driven phase transitions to figure out how to avoid explosive nucleation growth and cavitation induced shock wave damage. This discovery is now the heart of a major international effort involving over 50 surgeons to get this new concept into medical practice. It promises to take surgery from cold instruments to the ultimate (single cell) limit without risk of collateral damage – a veritable quantum leap in surgery. If the first atomic movie can lead to these kinds of potential societal benefits what can atom gazing bring as we look deeper into how chemistry and biology work at the atomic level? The future of this emerging field is “bright”.
This website will give you some insight into the group’s past and present activities, what we hope to contribute to our fundamental understanding of how things work at the atomic level, and where this new knowledge may lead under the research page.