Coherent Control and Multidimensional Spectroscopy of Complex Systems
Do we live in a Quantum World? Quantum mechanics dictates that all matter has an inherent wave property. On a molecular scale, this property can lead to destructive and constructive interferences that have a pronounced effect on transmission probabilities along reaction coordinates, for example the photo-induced isomerization of the retinal molecule in rhodopsins. Our goal in this lab is to explore whether quantum coherences can persist in nature such that pure quantum or phase related interference effects could play a role in functional responses. The timescales for electronic and nuclear decoherences are on the 10 to 100 fs time and 100 fs to ps time scales, respectively. This time scale is within the domain of barrier crossing dynamics to which proteins/enzymes have evolved to control barrier heights. The time and length scales of the underlying time dependent matter waves are consistent with quantum interference effects. The question reduces to the relevant decoherence timescales in relation to the barrier crossing dynamics leading to energy transduction and functions. We have investigated several objects, including dyes in solvents (artificial systems) and bacteriorhodopsin (a natural system) to answer this question.
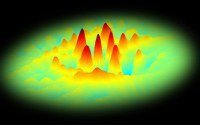
Our initial test system was Rhodamine 101 in methanol, where we were able to control the degree of population transfer from the ground to the excited state using laser pulses with specifically shaped phase and amplitude profiles at weak excitation where the response is linear with respect to the absorbed energy (see Prokhorenko et al J. Chem. Phys. 2005). Using a home-built coherent control setup (and computer-based feedback loop, after the methodology of Judson and Rabitz) we found that the temporal profile of the optimal pulse shape consists of a series of subpulses spaced apart by ~ 150 fs. Further, the optimal spectrum consists of a series of peaks spaced apart by 6.5 nm, corresponding to a frequency of 220 cm-1. This matches the frequency modulation of 150 fs in the population kinetics observed using pump-probe spectroscopy, indicating that even such large systems with many degrees of freedom are sensitive to constructive and destructive interference pathways created by shaped excitation pulses. The current interpretation is that the rhodamine 101 S0→S1 population transfer can be manipulated by keeping the underlying vibrational states in a coherent superposition during the excitation time period, thus modifying the resonant excitation (via time dependent Franck Condon factors that weight the transition probability). Similarly in bacteriorhodopsin, random fluctuations among the enormous number of degrees of freedom of protein might be expected to cancel any quantum interference effects. However, proteins are highly evolved structures and the question arises whether the phases of the underlying matter waves could play a role or even be manipulated in directing biological processes. To answer this, we have performed an experimental test of the possibility to manipulate the isomerization quantum yield of retinal in bacteriorhodopsin, a key molecule in light harvesting and vision, at very weak excitation levels (relevant to natural biological conditions) using a coherent control approach (see Prokhorenko et al Science 2006, Prokhorenko et al Chem. Phys. 2007). By manipulating the shape of the ultrashort laser pulse that jump-starts retinal's isomerization, we were able to enhance or suppress retinal isomerization yield by 20% in either direction and found the effect depends on the phase of the light field or timing of the various excited nuclear motions. The latter effect is consistent with constructive and destructive wave interference effects along the reaction coordinate defined by the protein structure. This experimental observation illustrates that the wave properties of matter can play a role in biological processes to the point that they can even be manipulated. This work also revealed the fundamental differences in coherent control and measurement for closed and open quantum systems. This work is all the most remarkable in that this fundamental bit of physics was unearthed using a biological system as the quantum object or test bed.
The unusual pulses shapes found to give rise to optimal and anti-optimal yields in the photoisomerization have now been rationalized using 2D spectroscopies in which we have directly observed strong vibrational nonadiabatic coupling (Johnson et al to be submitted). We have also developed a true optical analogue of NMR in which we can manipulate electronic/vibrational coherences in much the way spin coherences are manipulated in NMR to dissect structures (see Miller et al Acc. Chem. Res. 2010). Here we can dissect reaction dynamics and quantum interference effects. The merger of Coherent Control with 2D Spectroscopies made possible by the group’s development of diffractive optics for passive phase locking is now a truly Coherent Multidimensional Spectroscopy that enables one to fully resolve quantum state dynamics as well as manipulate them through the phase dependent terms in the propagation of the wavefunction propagation on the excited state surface (prior to the onset of decoherence in the vibrational modes coupled to reaction coordinates).
This methodology provides information on the electronic degrees of freedom. The ultimate goal is to understand how changes in electron distribution affect the interatomic forces, giving rise to the ensuing excited state dynamics and photoinduced chemical processes. Within the conventional approach using the Born-Oppenheimer (BO) approximation to think about time dependent molecular wavefunctions, the wavefunction is factored into electronic and nuclear wavefunctions. The coherent multidimensional spectroscopy gives the information on the electronic state dynamics. Ideally, one would like a direct measure of the nuclear wavefunction to fully understand the problem. The group has developed ultrabright electrons sources that literally light up atomic motions and give directly the nuclear motions – and by the time dependent velocities the very forces involved (see section on Femtosecond Electron Diffraction). With Coherent Multidimensional Spectroscopy and Femtosecond Electron Diffraction, we now can fully characterize time dependent molecular wavefunctions. The breakdown in the BO approximation for example occurs during chemical reactions involving conical intersections. At this point, there is strong vibrational nonadiabatic coupling to the electronic states such that we can no longer clearly separate the electronic and nuclear degrees of freedom. This occurrence appears to be more general that previously thought (or would like). The ability to directly observe the far from equilibrium atomic motions in this region provides the missing information needed to fully understand the wavefunction propagation in this region – and will greatly help theoretical methods come to first principle understanding of this key chemical process.
Marriage of Coherent Control/Multidimensional Spectroscopy with Electrons
Although Coherent Control methodology provides an important test for quantum coherence (especially in regards to quantum effects in biological systems), it is not possible to invert the optimized pulse shapes to retrieve the time dependent wavefunction (or phase information of the evolving wavefunction), save potentially for few atom systems (with a known ground state). This problem is yet another example of the classic problem in inverting complex functions. With a combination of Coherent Control strategies and femtosecond electron diffraction, it will be possible to directly observe the quantum interference and connect to specific nuclear motions. We will soon be able to directly observe the light matter interaction and dispel the “ball and stick” picture used to depict atomic motions – for a direct observation of matter waves.
Dynamic Structure of Liquid H2O
One of the long term objectives of the group is to experimentally determine the many body potential of liquid H2O – the “liquor of life”. This interest is a natural extension of our interest in biological systems and solution phase chemistry. To this end, two new spectroscopic methods have been developed based on diffractive optics. This single advance has led to phase stabilized pulse sequences that can be generated from a single optic. This development solved a long standing problem in optical analogues of NMR with respect to generating phase locked pulse sequences (see Cowan et al Chem. Phys. Lett. 2004, Goodno et al J. Opt. Soc. Am. B: Opt. Phys. 1998, Miller et al. Acc. Chem Res. 2010). This development in turn has made it possible to routinely execute heterodyne detection in which the signal to noise is typically increased over a factor of 100 over homodyne or intensity based detection methods. This increased sensitivity and ability to encode even complex beam patterns with the desired phase matching beam geometries using diffractive optics opened up the field of high order nonlinear spectroscopy – namely 6-wave mixing processes. Each different nonlinear interaction probes a different aspect of either electronic or nuclear degrees of freedom that lead to direct determinations of the operating decoherence time scales in the frequency correlations (or memory) of the degree of freedom on interest as well as anharmonic coupling to other degrees of freedom. This information is exactly that needed to build up a first principles understanding of the dynamic structure of liquids and other complex systems. Two different spectral domains have been explored in this context.
The use of 2D-IR spectroscopy gives an unique probe into coupling between vibrational modes and inhomogeneous/homogeneous broadening and dynamics. The OH stretch region of liquid water provides a direct probe of the hydrogen bond network giving rise to water’s unique properties. Unfortunately (or fortunately as we discussed below with respect to laser surgical applications), the absorptivity of water is so high in this region that pathlengths on the 100 nm scale are needed to remain in the low OD limit for nonlinear spectroscopy. Prior to the group’s work, it was not possible to study pure H2O. Studies defaulted to isotopic variants with off resonant OD probes of the hydrogen bond structure. The development of diffractive optics and more importantly for this specific problem, the development of nanofluidic cells for this problem made it possible to study the fully resonant network of pure H2O (see Cowan et al Nature 2005, Kraemer et al PNAS 2008). We found the remarkable result that water loses its so-called memory in less than 100 femtoseconds, more than an order of magnitude faster than any other liquid. The degree of hydrogen bonded played an extremely important role. Relative small changes in lowering the temperature and increasing the degree of hydrogen bonding led to dramatic increases in the coherence time of the OH stretch. The strong dipole-dipole coupling between waters leads to spatial migration of the excitation that is actually faster than the decoherence time. The picture that has emerged is that the OH stretch in pure water is not localized on a single water but is rather spatially delocalized forming a vibrational exciton in which the number of waters coherently involved in the effective vibrational mode(oscillating dipole and induced polarization) depends sensitively on temperature and spatial coherence within the hydrogen bond network. These extraordinarily fast dynamics, spatial coherence, and energy redistribution in water’s hydrogen bond network have profound implications for biological systems.
We now have a completely different model for liquid water from that of a localized basis. It is also clear that the current level of ab initio theory for liquid water is insufficient as the calculations must include > 20 waters in an exact manner just to capture the vibrational mode coupling leading to delocalized vibrational excitons and energy redistribution. We are in the process of developing new tools to provide a direct observation of the structural correlations in liquid water based on high coherence electron and x-ray sources (XFELs) made possible with recent advances in nanofluidics by the group (see material section).
Six-Wave Mixing: χ(5) Studies of Liquids
The above studies focus on the high frequency intramolecular vibrations to report on liquid dynamics. The key motions typically involved in reaction dynamics are the low frequency intermolecular motions, involving unknown spatial correlations. Similar issues arise in understanding protein dynamics, however, the spatial correlations are imposed by the specific structure of the protein. How can we probe these low frequency motions? To this end, the group developed diffractive optics based 2D fifth-order Raman spectroscopy specifically to the study of the low frequency collective motions of liquids. This relatively new form of spectroscopy uses electronically nonresonant Raman processes to selectively probe the anharmonic terms in the relative intermolecular motions. It is arguably one of the most difficult nonlinear experiments ever conducted. In the 6-field interaction, there is a rephasing pathway between the excitation pulse pairs (4 excitation pulse sequence) that involves a 2-quanta transition or Raman overtone. The large number of beams that must be properly phase matched and small signal makes the experiment quite challenging. However, the reward is in the high sensitivity of the observable to the details of the intermolecular potential. The dephasing of the induced low frequency intermolecular motions of liquids through the Raman response is dominated by the anharmonic terms in these motions. This feature of this spectroscopy is particulary important. The one distinguishing feature of a liquid from say a glass is the relative anharmonic motions on essentially all time scales that lead to the distinctive macroscopic property that liquids do not support shear (as discussed above in the context of femtosecond electron diffraction studies of melting dynamics). However, there is an inertial phase of the various intermolecular motions that separates the time scale of Brownian or diffusive motion; on short enough time scales, long range correlations in liquids are still conserved and one can think of a modal basis to describe these motions. This new form of spectroscopy gives the anharmonic couplings between this collective mode basis and in this sense builds up the many body potential.
This experiment is really the proving ground for both theory and experiment on the liquid state. It has led to new methods of using phase matching, phase cycling, and polarization contrast for signal detection, as well as new laser technologies to access the required pulse durations and peak power. The research to date has focused on simple liquids such as CS2, benzene, and most recently the highly hydrogen bonded structure of formamide as a model system for beta sheets in biological systems (see Kubarych et al J. Chem. Phys. 2002, Milne et al J. Phys. Chem. B 2006, Li et al). We have also built up capabilities in MD calculations of the expected response at the χ(5) level on interaction in which we can explore the sensitivity of the liquid response to the details of assumed intermolecular potential. This aspect of the work has shown how sensitive this form of spectroscopy truly is. It is likely that χ(5) responses will form the most rigorous benchmark for testing intermolecular potentials. The problem in extending this work to water is that the expected signal from water is still an order of magnitude smaller than formamide (upper limit confirmed experimentally).
The above discussion is to illustrate the group’s long standing interest in liquid state dynamics and our contributions to this field. Nearly all of chemistry occurs within solution phase or mixed phases and all of biology is based on aqueous chemistry. The lack of any long range order and extraordinarily fast timescales governing the primary processes in the liquid state make this problem challenging. However, this problem is of central importance to understanding chemistry and needs to be pursued to the space-time limits governing chemical processes. The next phase of the research will focus on using electron probes of liquid phase dynamics. The nanofluidic cell concept developed for the study of pure liquid H2O has paid off enormous dividends in terms of opening up the study of liquid dynamics now with femtosecond electron probes. The major push in this direction is to use the new electron sources in combination with our nanofluidic cell technology to enable atomically resolved dynamics in the solution phase. In this pursuit, we have the distinct possibility of directly observing the low frequency modes of the liquid coupled to reaction coordinates through changes in the nearest neighbor pair correlation function, as probed by diffraction methods. This is one of the most exciting new directions for the group in that it is now touching upon arguably the most important problems in chemistry – the role of the bath in chemical reactions.